This introductory paper is the research content of the paper "High Temperature Strength Reduces Soldering In Aluminum High Pressure Die Casting" published by Open Access Dissertation.
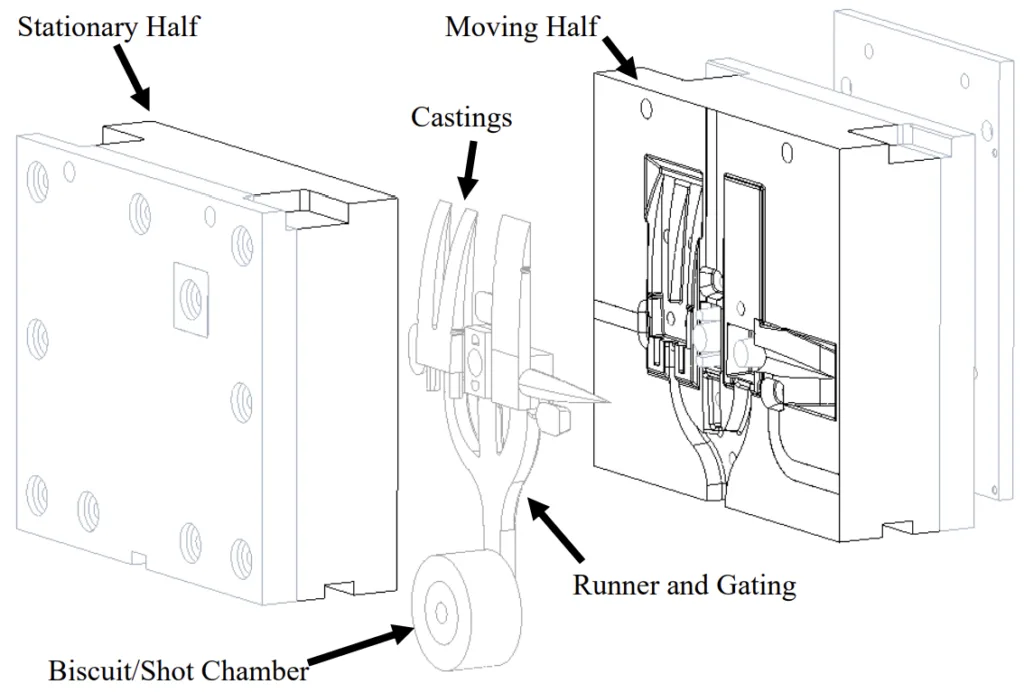
1. Overview:
- Title: High Temperature Strength Reduces Soldering In Aluminum High Pressure Die Casting
- Author: Jacob A. Belke
- Publication Year: 2024
- Published Journal/Society: Open Access Dissertation, Michigan Technological University
- Keywords: Die Casting, Soldering, Aluminum Alloys, High Temperature Strength, Tresca Friction, Skin Formation, Magnesium Silicide, Orowan Strengthening
2. Abstract
Die soldering, an adhesion defect in high pressure die casting (HPDC), is a symptom of localized sticking where a localized portion of the cast material is adhered to the tooling surface causing build up over time. This requires the tooling to be serviced which incurs additional costs to the process that gets passed on to the parts. Historically, soldering has been mitigated using lubricants, coatings, and alloy chemistry modifications but solder persists.
The Tresca friction thermomechanical model suggests soldering occurs when the local interfacial shear stress between the casting and die surface exceeds the local shear strength of the casting. The ratio of these shear strengths, as a function of temperature, has been shown to predict solder. Research up to this point has focused on reducing the friction coefficient, and in turn the interface shear strength, with no work done on the strengthening of the castings regarding solder. Chemistry of the alloy has been shown to influence soldering behavior, but for the wrong reason as Al-Fe intermetallics are the commonly accepted soldering mechanism.
High temperature strengthening mechanisms through chemistry modifications were investigated to support the Tresca friction model. First, the improvement of the solid solution and Orowan strengthening mechanisms was quantified for several aluminum HPDC alloys through the addition of magnesium, improving the hot shear strength of the alloys. Next, the improved alloy shear strength was applied to the Tresca model and tested using a laboratory scale permanent mold designed to solder along with a full scale HPDC production trial with results pointing towards a new soldering mechanism. Finally, the relationship between solder and the casting surface chill zone or “skin” is examined and discussed.
3. Research Background:
Background of the research topic:
Aluminum high pressure die casting (HPDC) is a widely used process for producing high-strength, thin-walled castings, but it is plagued by die soldering, where the cast alloy adheres to the die surface. This necessitates costly tool maintenance and reduces productivity.
Status of previous research:
- Traditional approaches to mitigate soldering include using die coatings, lubricants, and high iron content alloys, with limited success.
- The historically accepted mechanism for soldering is the formation of Al-Fe intermetallic compounds.
- Kinetics analysis, shows that the intermetallic compound growth is to slow to cause the soldering during one HPDC shot. (Figure 2.1)
-Aluminizing hot dip tests have established that growth of the AlxFey intermetallic phases is governed by chemical reactions at the interfaces resulting in linear kinetics [22].
-Shankar and Apelian’s core pin dip test found it took about 100 seconds for intermetallics to grow 100 μm [27].
-Experiments from Terek et al. found that a buildup of 100 μm on a core pin was possible in just one diecast cycle [28]. - Recent research has introduced a thermomechanical model (Tresca friction) that considers the ratio of interfacial shear stress to the casting's shear strength.
- Prior work has focused on reducing the friction coefficient, but not on increasing the casting's strength.
Need for research:
Existing soldering mitigation strategies are insufficient. A deeper understanding of the relationship between alloy composition, high-temperature mechanical properties, and the soldering phenomenon is needed to develop more effective solutions.
4. Research purpose and research question:
Research purpose:
To investigate the dependency of the strength of HPDC aluminum alloys on adhesion and solder formation, focusing on high-temperature strengthening mechanisms and their impact on the Tresca friction model.
Core research:
- Quantify the effect of magnesium additions on the shear strength of aluminum-silicon alloys at elevated temperatures.
- Apply the thermomechanical Tresca model to a casting designed to solder.
-Conduct a production HPDC trial of a Tresca optimized alloy chemistry.
-Examine the skin and its relationship with die soldering.
5. Research methodology
- Research Design: Experimental, using a combination of laboratory-scale testing and a full-scale production HPDC trial.
- Alloy Preparation: Al-Si-Mg (A356, A362) and Al-Si-Cu (A380) alloys were prepared with varying magnesium concentrations (up to 1.0 wt%). (Table 4.2)
- Data Collection:
- Hot Shear Testing: A modified Amsler shear test was used to measure the shear strength of the alloys at temperatures ranging from 20-500°C. (Figure 4.7)
- Permanent Mold Soldering Experiment: A custom-designed permanent mold with a stepped D-shaped core pin (Figure 5.1) was used to induce soldering under controlled conditions.
- HPDC Production Trial: A 100-shot production trial was conducted using A380 and A380Mg0.6 alloys in a powersport engine case casting. (Figure 6.3)
- Microscopy: Optical and scanning electron microscopy (SEM) with EDS were used to analyze the microstructure and composition of the castings. (Figure 6.23, 6.24, 6.25)
- TEM Foil Preparation and Imaging: Brightfield TEM images and STEM-EDS maps were taken using a FEI Themis Z STEM at 200 kV with a calculated probe size of 1.4 nm. (Figure 4.12, 4.13)
- Analysis Method:
- Tresca friction criteria were calculated using measured shear strengths, contact pressures (from MAGMASOFT simulations), and friction coefficients.
- Statistical analysis (paired t-tests, ANOVA) was used to evaluate the significance of the results.
-Image J analysis was used to analyze the Optical microscopy and TEM images.
- Research Scope: The study focused on aluminum HPDC alloys, specifically A356, A362, and A380, with a focus on the effects of magnesium additions.
6. Key research results:
Key research results and presented data analysis:
- Hot Shear Testing:
- Increasing magnesium content significantly increased the shear strength of A356 and A380 alloys at elevated temperatures. (Figure 4.8, 4.10)
- A380Mg1 exhibited the highest shear strength among the tested alloys.
- A362 showed no increase with increased Mg. (Figure 4.9)
- Permanent Mold Soldering Experiment:
- Soldering behavior generally aligned with Tresca friction model predictions.
- A356 exhibited more severe soldering than A380.
- Magnesium additions reduced soldering in all alloys.
- HPDC Production Trial:
- A380Mg0.6 showed significantly reduced soldering compared to A380. (Figure 6.8, 6.9, 6.10)
- Solder formation correlated with areas of lower cooling rates and the absence of a well-defined "skin" layer.
-Solder formation correlated with areas of thinner skin formation. (Figure 7.8)
- Microscopy:
- A distinct "skin" layer with a fine-grained, porosity-free microstructure was observed in areas with high cooling rates. (Figure 7.1)
- Soldering was associated with mechanical deformation and failure in the weaker matrix material beneath the skin. (Figure 5.13)
- TEM:
-A380 showed a dislocation Al2Cu precipitate interaction. (Figure 4.12)
-A380Mg1 showed a dislocation- Al3Cu2Mg9Si7 precipitate interaction. (Figure 4.13)
List of figure names:
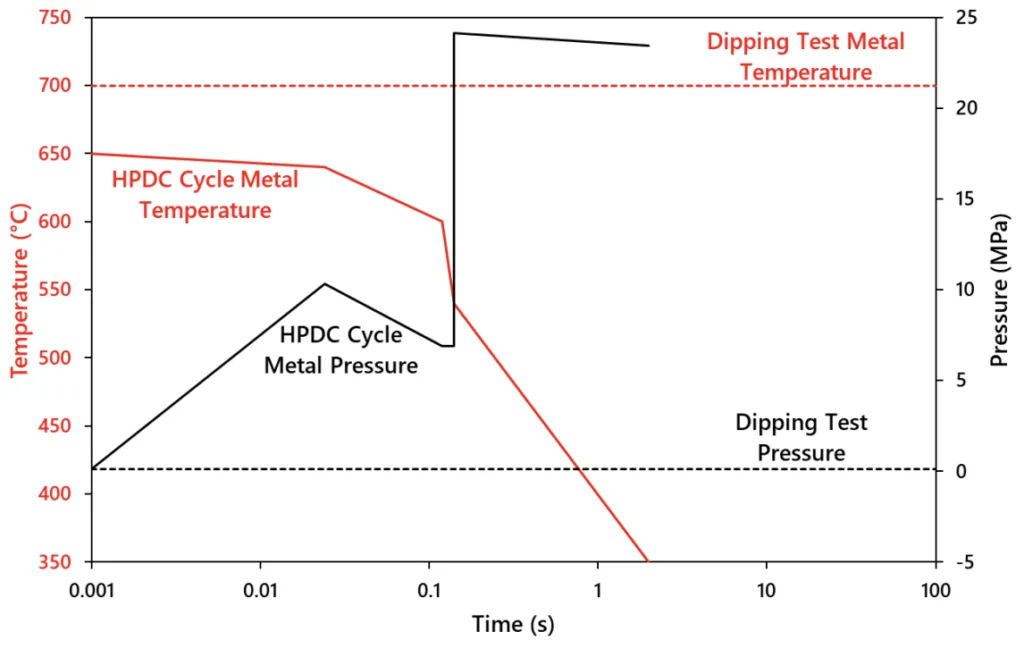
![Figure 2.3: Phases precipitated out during solidification as a function of iron content and
cooling rate [47]. The Al-Fe-Si ternary eutectic composition occurs at about 0.8% Fe.](https://castman.co.kr/wp-content/uploads/image-1534-1024x702.webp)
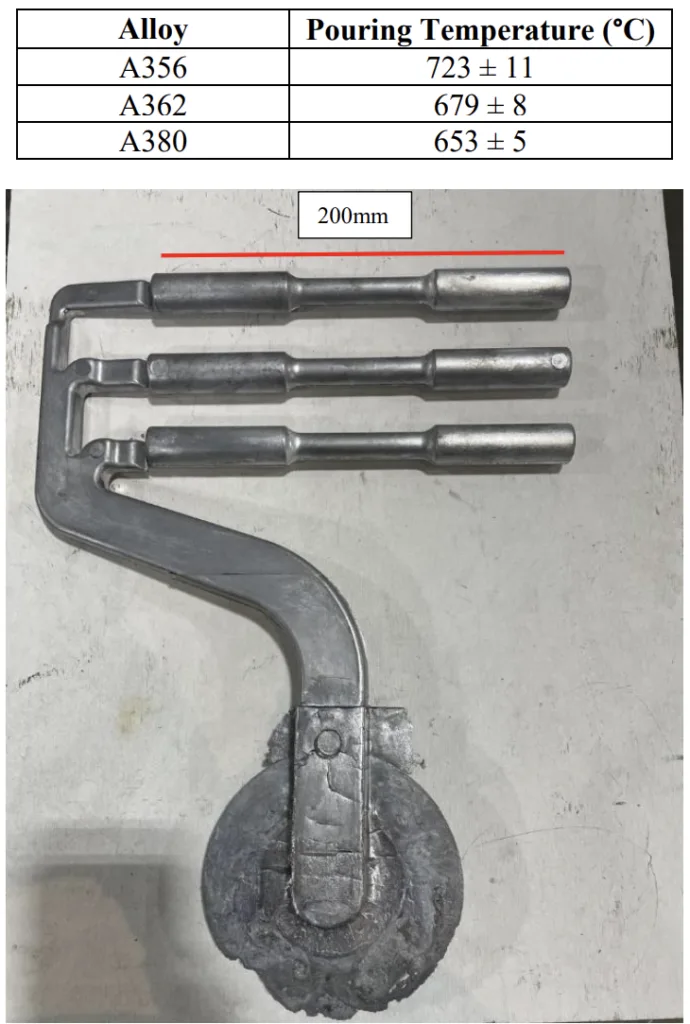
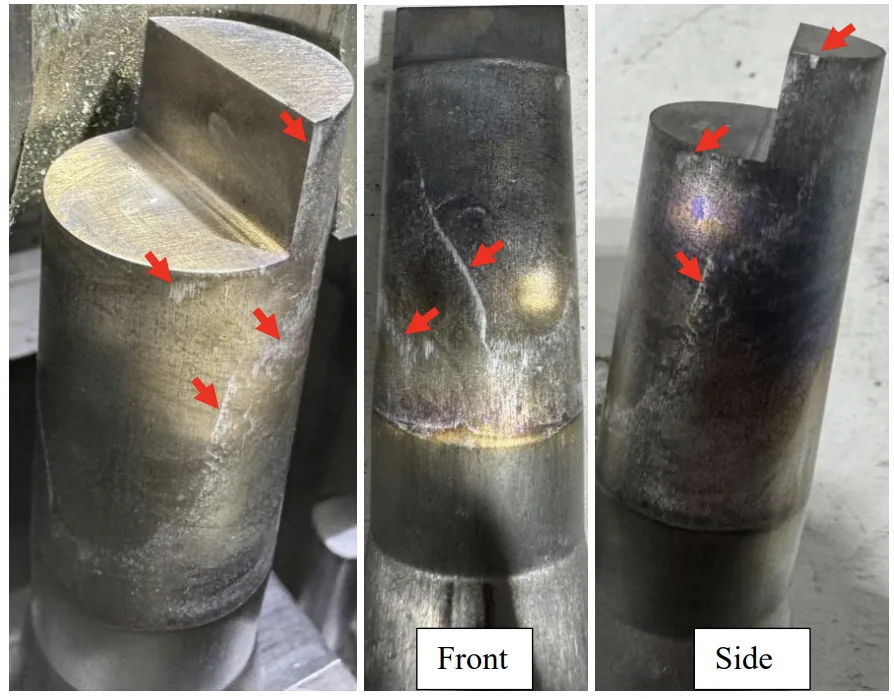
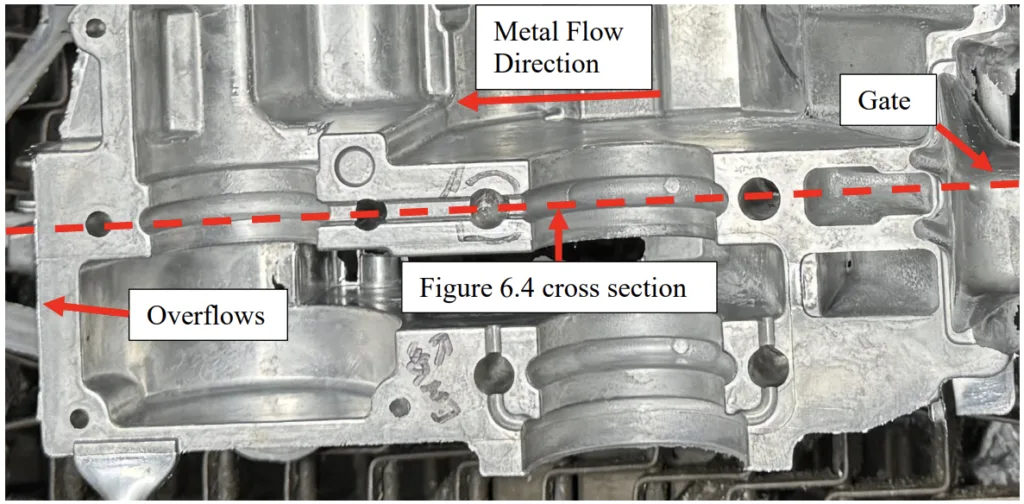
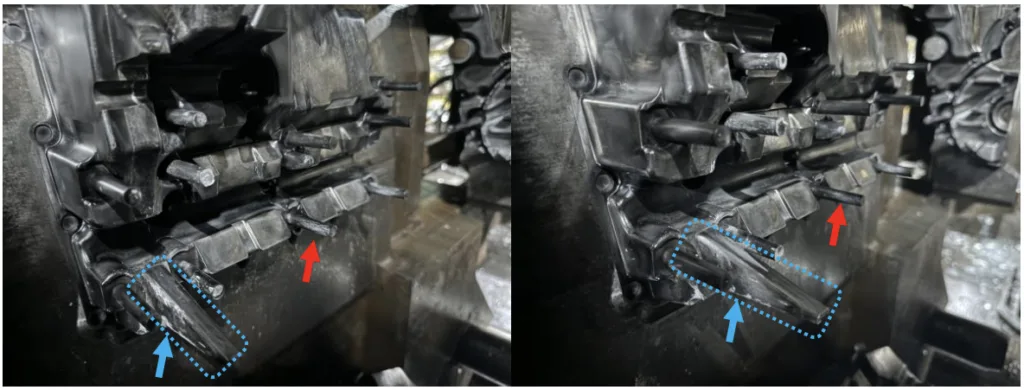
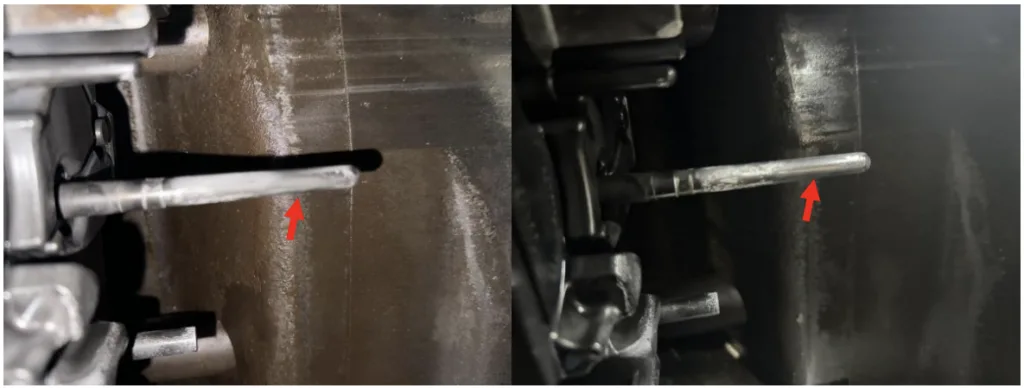
- Figure 1.1: Example model of a HPDC die cavity.
- Figure 1.2: High pressure die casting tooling pulled from production due to solder on the core pin (silver material circled).
- Figure 2.1: Plot of the intermetallic growth rate observed in dip tests at 625°C [27] and HPDC trials [28].
- Figure 2.2: Comparison of the typical test conditions of temperature (red) and pressure (black) of dip tests (dashed) and HPDC (solid). The time scale was set to a typical HPDC filling cycle. Dip tests continue at these constant conditions for minutes to hours.
- Figure 2.3: Phases precipitated out during solidification as a function of iron content and cooling rate [47]. The Al-Fe-Si ternary eutectic composition occurs at about 0.8% Fe.
- Figure 2.4: Plot of the anti-soldering tendencies of various alloys along with their typical UTS (yellow) and yield (orange).
- Figure 2.5: Plot of the anti-soldering tendencies of various alloys along with their typical elongations (red).
- Figure 2.6: Darken-Gurry plot of electronegativity vs metallic radius for a coordination number of 12 (FCC). The ellipse centered around the red Al point has a major axis of ±0.4 electronegativity unit and a minor axis of ±15% of the metallic radius. The tangent lines show the simple ±15% Hume-Rothery size rule.
- Figure 2.7: Phase change diagram for aluminum and Al-12.6% Si from liquid to solid. The length of the horizontal segment corresponds to the value of the enthalpy of fusion. The slope of the cooling lines is controlled by the heat capacity of the elements.
- Figure 2.8: Solid solution strengthening of Mg in pure Al, 0.5% strain compression [63].
- Figure 2.9: Effect of magnesium content on the UTS of Al-Si alloys [70], [71], [72].
- Figure 2.10: Visual representation of Schmid’s Law showing a tensile force applied to a single crystal and the resulting slip system.
- Figure 2.11: Example of a single crystal of aluminum on a steel core pin during ejection. The ejection force results in slip occurring before the UTS.
- Figure 2.12: Schmid's Law plotted as a function of crystal orientation observed in single crystals of magnesium [87].
- Figure 3.1: Experimentally measured yield strength of A380 from ASM Handbook converted to shear strength using Equation 6 (black), postulated 10% increase in shear strength of A380 with increased Mg (green), and the postulated interface shear strength (black dash) from 0°C to 600°C [90].
- Figure 4.1: Simple cubic drawing of an edge dislocation (left) and screw dislocation (right), adapted from [92].
- Figure 4.2: Depiction of lattice distortions caused by substitutional and interstitial atoms, adapted from [92].
- Figure 4.3: Solid solution hardening potential for Mg, Cu, and Si in Al. Strengths are plotted up each element’s solubility limit. Data for the scaling factor from [96].
- Figure 4.4: Temperature dependency of solid solution hardening for Mg and Cu in Al. The solute content was found to insignificantly impact on the temperature dependency, so a solute content of 1 at% for Mg and Cu was modeled for simplicity.
- Figure 4.5: Tensile bar die casting in which hot shear specimens were taken from the middle of the reduced section of the tensile bars.
- Figure 4.6: X-ray image of shear specimen showing a porosity free center testing region. The light regions at either end of the specimen are bores for the insertion heaters.
- Figure 4.7: Hot shear testing using Amsler shear tool and PID controlled insertion heaters.
- Figure 4.8: Effect of Mg content on the shear fracture strength of A356 in the as-cast condition over a temperature range of 20-500°C.
- Figure 4.9: Effect of Mg content on the shear fracture strength of A362 in the as-cast condition over a temperature range of 20-500°C.
- Figure 4.10: Effect of Mg content on the shear fracture strength of A380 in the as-cast condition over a temperature range of 20-500°C.
- Figure 4.11: Precipitate hardening potential for Al2Cu (θ″). A precipitate diameter of 20 nm was used for the calculation based on measurements from Figure 4.12.
- Figure 4.12: BF-STEM image of A380 and the corresponding Cu (blue) and Si (green) EDS elemental maps. The arrows on the EDS maps show the Al2Cu (θ″) and Si precipitates. The BF-STEM image shows an accumulation of pinned dislocations (dark lines) against the top left Si plate and bottom right Al2Cu (θ″).
- Figure 4.13: BF-STEM image of A380Mg1 and the corresponding Cu (blue), Si (green) and Mg (yellow) EDS elemental maps. The Al3Cu2Mg9Si7 (Q/Q′) precipitates, indicated by the arrows on the Si EDS map, are shown to have precipitated on a grain boundary. The BF-STEM image identifies a free dislocation and an Orowan pinned dislocation by a Q/Q′ precipitate where the dislocation has started to bow.
- Figure 4.14: Orowan strengthening contribution from Q/Q′ precipitates in A380Mg1 using the mean spacing between precipitates from Figure 4.13. Calculated shear strength (185 MPa) is lower than the measured value (223 MPa) in Table 4.4. Including the contribution from solid solution strengthening (Figure 4.3), the shear strength is estimated to be 213 MPa at room temperature.
- Figure 4.15: Scheil solidification calculation comparing A380 and A380Mg1 precipitates from 0.7 to 1.0 fraction solid.
- Figure 4.16: Scheil solidification calculation comparing A356 and A356Mg1 precipitates from 0.7 to 1.0 fraction solid.
- Figure 4.17: Scheil solidification calculation comparing A362 and A362Mg1 precipitates from 0.7 to 1.0 fraction solid.
- Figure 5.1: Stepped D-shaped core pin used in solder permanent mold trials. The core pin has a draft angle of 2°, step height of 10 mm, and polished to a 220-draw stone polish.
- Figure 5.2: Centerline of permanent mold soldering experiment. The die and core pin were made of uncoated H13-tool steel. Note the casting fills on the tall or back side of the core pin.
- Figure 5.3: Contact pressure map of four core-pin regions of interest at 200°C when the maximum pressure occurs. , 380,200℃ = 75 .
- Figure 5.4: Contact pressure plotted for four regions of interest in Figure 5.3 and yield strength of the casting over the temperature range of 600-200°C. The casting will yield at locations 1 and 4 during solidification.
- Figure 5.5: Tresca friction criteria for A380 and A380Mg1 from 20-500°C in which values below dashed line are predicted not to stick of solder.
- Figure 5.6: Tresca friction criteria for A356 and A356Mg1 from 20-500°C in which values below dashed line are predicted not to stick of solder.
- Figure 5.7: Tresca friction criteria for A362 and A362Mg1 from 20-500°C in which values below dashed line are predicted not to stick of solder.
- Figure 5.8: Ejection force vs ejection temperature from 300-500°C. Error bars are 95% standard error of the mean.
- Figure 5.9: A356 core pin ejected at 400°C. Red arrows indicate solder. The core pin has a diameter of 25mm.
- Figure 5.10: A362 core pin ejected at 400°C. Red arrows indicate solder. The core pin has a diameter of 25mm.
- Figure 5.11: A380Mg1 core pin ejected at 500°C. Red arrows indicate solder. The core pin has a diameter of 25mm.
- Figure 5.12: A356Mg1 core pin ejected at 500°C. Red arrows indicate solder. The core pin has a diameter of 25mm.
- Figure 5.13: Cross section of A356 400°C casting trial showing solder scars from ejection.
- Figure 5.14: Cross section of A356 400°C casting showing mechanical deformation zone underneath solder scars from ejection.
- Figure 6.1: MAGMA simulation result of a clipped section from the overall casting comparing results for die soldering (left) and contact pressure at ejection (right). Higher times in the die soldering result (left) and higher contact pressures (right) indicate solder prone areas.
- Figure 6.2: Tresca friction criteria calculation for location 2 in Figure 6.1 comparing A380, A380Mg0.4, and A380Mg0.6.
- Figure 6.3: Bottom view of HPDC engine cover casting used in trial. Casting was sectioned across the red dashed line for microscopy (Figure 6.4).
- Figure 6.4: Cross section of casting with microscopy locations annotated. The red crosshatched area is the microscopy viewing plane.
- Figure 6.5: Moving half of die before the trial (left) and after 100 shots of A380 (right). Severely soldered core pins are identified with red arrows. Yellow arrows annotate areas used for solder comparison (Figure 6.7). Area A is on the moving die half and B is on a slide.
- Figure 6.6: Moving half of die before spray (left) and after die lube spray (right). Black arrows annotate areas used for solder comparison (Figure 6.7). Area A is on the moving die half and B is on a slide. Temperature is upper left is of the crosshair region, which is the same location for both images.
- Figure 6.7: Comparison of soldering behavior of A380 between shot 1 (left) and shot 100 (right) for three different areas (Figure 6.5). Note the gates (top image) are in the stationary half of the die which is not pictured in Figure 6.5. Red arrows point to solder which is silver/white.
- Figure 6.8: Moving half of die after 100 shots of A380 (left) and after 100 shots of A380Mg0.6 (right). Red and blue arrows indicate two examples where the solder was reduced on the core pin from the control run to the Mg addition run.
- Figure 6.9: Core pin after 100 shots of A380 (left) and after 100 shots of A380Mg0.6 (right). The A380Mg0.6 core pin is visibly cleaner than the A380 with less solder at the tip and base.
- Figure 6.10: Stationary die after 100 shots of A380 (left) and after 100 shots of A380Mg0.6 (right). The A380Mg0.6 die is visibly cleaner (less solder) than the A380 (red arrows).
- Figure 6.11: Comparison of soldering behavior of A380Mg0.6 between shot 1 (left) and shot 100 (right) for two different areas (Figure 6.5). Red arrows point to solder which is silver/white.
- Figure 6.12: Cooling rate map for the horizontal cored hole (Figure 6.4).
- Figure 6.13: Orowan strengthening calculation for blocky Si in Figure 6.17 and Figure 6.21 bottom and rod eutectic Si in Figure 6.17 top and fine blocky Si in Figure 6.21 (Equation 19). Mean particle spacing measured using ImageJ 1.54i software. Large blocky Si particles at the bottom do not contribute significantly to the Orowan strengthening of the casting.
- Figure 6.14: A380 vertical cored hole micrograph at increasing magnification. Blocky Si at the surface transitions to eutectic Si 200 μm from the surface along with the formation of porosity.
- Figure 6.15: Higher magnification of Figure 6.14 showing blocky Si close to the core pin surface (top) and coarse eutectic Si further into the casting (bottom).
- Figure 6.16: Mosaic of horizontal cored hole with orientation relative to Figure 6.4 showing the solder scar at the bottom of the cored hole where the matrix is weaker.
- Figure 6.17: A380 top (a) and bottom (b) of the horizontal cored hole in Figure 6.16 showing a difference in the scale of microstructure and Si morphology. A skin is present in the top of the cored hole but is absent in the bottom.
- Figure 6.18: A380 surface feature micrograph in Figure 6.4. Skin is absent in this location compared to the vertical and horizontal cored holes along with Al-Si eutectic and intermetallics are present at the surface indicating a slower cooling rate.
- Figure 6.19: A380Mg0.6 vertical cored hole micrograph at increasing magnification. Blocky Si at the surface transitions to eutectic Si 525 μm from the surface.
- Figure 6.20: Higher magnification of Figure 6.19 showing blocky Si close to the core pin surface (top) and eutectic Si further into the casting (bottom).
- Figure 6.21: A380Mg0.6 horizontal cored hole micrograph at the top (a) and bottom (b) of the horizontal cored hole. Very fine blocky Si is found at the top surface of the cored hole. A skin layer is absent from both surfaces.
- Figure 6.22: A380Mg0.6 surface feature micrograph in Figure 6.4. Very fine Al-Si rod eutectic present at the surface indicating a fast-cooling rate. The large sludge particles precipitated in the ladle or shot chamber when the melt temperature dropped below the sludge factor (Equation 3) and were transported near the skin during filling.
- Figure 6.23: A380 backscatter electron image of horizontal cored hole top (top) and an EDS map overlay (bottom) with Si (green), Cu (blue), Mg (yellow), Fe (pink), and the dark region is the Al matrix. Si and Cu coarsen further away from the surface. Fe precipitates are nearly absent within 100 μm of the surface.
- Figure 6.24: A380 backscatter electron image of horizontal cored hole bottom (top) and an EDS map overlay (bottom) with Si (green), Cu (blue), Mg (yellow), Fe (pink), and the dark region is the Al matrix. Blocky Si and Cu highlight the interdendric regions with fine Fe precipitates near the surface and sludge particles 100 μm from the surface.
- Figure 6.25: A380 backscatter electron image of horizontal cored hole matrix (top) and an EDS map overlay (bottom) with Si (green), Cu (blue), Mg (yellow), Fe (pink), and the dark region is the Al matrix. Eutectic Si and Cu are evenly dispersed throughout the matrix with a greater number of sludge particles compared to the surface.
- Figure 6.26: A380Mg0.6 backscatter electron image of horizontal cored hole top (top) and an EDS map overlay (bottom) with Si (green), Cu (blue), Mg (yellow), Fe (pink), and the dark region is the Al matrix. Si is the only element present in the 25 μm skin coarsening further away from the surface. Mg precipitates nucleated near the Si eutectic.
- Figure 6.27: A380Mg0.6 individual EDS maps of Figure 6.26 with Si (green), Cu (blue), Mg (yellow), Fe (pink), and the dark region is the Al matrix. Si is the only abundant element present within 25 μm of the surface.
- Figure 6.28: A380Mg0.6 backscatter electron image of horizontal cored hole bottom (top) and an EDS map overlay (bottom) with Si (green), Cu (blue), Mg (yellow), Fe (pink), and the dark region is the Al matrix. Large Cu and Mg precipitates are observed at the surface with less blocky Si within 50 μm of the surface.
- Figure 6.29: A380Mg0.6 EDS map of horizontal cored hole bottom Si (green), Cu (blue), Mg (yellow), Fe (pink), and the dark region is the Al matrix. The red arrow identifies the location of EDS point analysis.
- Figure 6.30: A380Mg0.6 backscatter electron image of horizontal cored hole matrix (top) and an EDS map overlay (bottom) with Si (green), Cu (blue), Mg (yellow), Fe (pink), and the dark region is the Al matrix. The Cu and Mg rich precipitates nucleated from the sludge particle. The Si eutectic structure is visible from a lower beam interaction volume.
- Figure 7.1: Optical micrograph of A380 with a 10-20 μm ultra-fine equiaxed skin layer that transitions to large equiaxed grains. Columnar grains are not observed. Etched with Keller’s reagent.
- Figure 7.2: Time to grow a 100 μm thick skin as a function of with various HTCs using Yuan's parameters [145].
- Figure 7.3: A380 (a) and A380Mg0.6 (b) EDS point analysis of α-Al locations 1 (blue), 25 (red), 50 (green) μm from the surface. Point sizes scaled to interaction volume.
- Figure 7.4: A380 EDS point results for 1 μm (blue), 25 μm (red), and 50 μm (green) from the surface. Error bars are 95% standard error of the mean from multiple spatial points. Only Cu and Zn have an ANOVA p-value < 0.05, making them statistically significant. -Figure 7.5: A380Mg0.6 EDS point results for 1 μm (blue), 25 μm (red), and 50 μm (green) from the surface. Error bars are 95% standard error of the mean from multiple spatial points. Only Cu and Zn have an ANOVA p-value < 0.05, making them statistically significant. -Figure 7.6: Scheil solidification of the skin and core for A380 and A380Mg0.6. The skin calculation considers solute trapping with a solidification interface velocity of 500,000 μm/s. The core calculation assumes the extreme of equilibrium during solidification. -Figure 7.7: Illustration of the HPDC skin (a) with three different separation scenarios between the steel die, skin, and aluminum under the shear condition of the ejection force, (b-d). (b) When the die:casting interface strength is lower than the strength of the skin layer and the aluminum matrix, a clean separation is observed. (c) Failure at the skin-matrix interface due to porosity and low strength of aluminum matrix at elevated temperature. (d) Shear strain field, , from ejection does not extend into the weaker aluminum matrix due to a thicker skin layer resulting in a clean ejection. -Figure 7.8: MAGMA simulation result from the casting in Chapter 6 (Figure 6.4). Skin thickness (called the pore free zone by MAGMA) (left) and die soldering (right) shows that solder is predicted in areas with thin skin (arrows). Simulation mesh cell size was set at 1 mm. Finer mesh sizes increase the resolution of the skin and solder predictions but come at the cost of simulation time and can quickly reach impractical times. -Figure 7.9: Close up of Figure 7.8. in which the skin thickness, or pore free zone (left) and die soldering (right) clearly shows that solder is predicted in areas with a thin skin; the top (green arrow) of core pin region is predicted to have a thicker skin than the bottom (red arrow), > .
- Figure 7.10: Infrared image of casting 10 seconds after ejection from Chapter 6 showing solder prone cored holes over 100°C hotter than solder free surface features.
- Figure 7.11: A380 horizontal cored hole micrograph at the top (a) with a 10-20 μm skin and bottom (b) of the horizontal cored hole with no skin. The bottom of core pin contains deformation zone underneath solder scars from ejection. Etched with Keller’s reagent.
- Figure 7.12: A380Mg0.6 horizontal cored hole micrograph at the top (a) and bottom (b) of the horizontal cored hole with no skin present. The bottom of core pin contains deformation zone at the surface along with a coarser microstructure and porosity. Etched with Keller’s reagent.
- -Figure 7.13: Progression of solder growth where thin individual layers are applied on top of each other to generate a thicker structure. The first shot deposits a skin which may adhere to the die similar to Figure 7.7(c). Subsequent shots may add additional skin layers on top of subsequent layers. Progressive layers are gradually thinner due to slower heat transfer between the water-cooled steel through the skin to the molten aluminum in the most recent shot.
- Figure 7.14: Microscopy sample location comparing skin thickness for each casting cycle. The casting was sectioned parallel to the plane of the step (black dashed line) with the viewing plane perpendicular to the step (solid red box). Casting is drafted at the top for ejection from the die.
- Figure 7.15: Ejection force and core pin starting temperature for A380 over six casting cycles. Solder formed on the ejection of the sixth cycle. Cycle 3 ejection force was not measured due to a loss of the thermocouple signal, so the ejection
- Figure 7.16: A380 core pin ejected at 300°C after six cycles with a starting temperature of 172°C. Red arrows indicate solder.
- Figure 7.17: Cooling rate for each casting cycle with gradually higher core pin temperature. Higher core pin temperatures lower the cooling rate as indicated by the regression slope. Cycle 3 was omitted due to a thermocouple measurement error during cooling.
- Figure 7.18: Optical metallographs (Figure 7.14) from each casting cycle showing skin thickness reducing in thickness and moving towards center of casting until elimination in cycle 5. The skin transition was defined by the change in morphology of the Si pro-eutectic from octahedral to the coarse needles. Solder formed after cycle 6.
7. Conclusion:
Summary of key findings:
- Magnesium additions significantly increase the high-temperature shear strength of aluminum HPDC alloys through solid solution and precipitation strengthening (Mg2Si and Al3Cu2Mg9Si7).
- The Tresca friction model, incorporating alloy shear strength, provides a good prediction of soldering behavior.
- A distinct "skin" layer forms on HPDC castings, characterized by a fine-grained, porosity-free microstructure and higher solute concentrations.
- Soldering is associated with failure in the weaker matrix material beneath the skin, particularly in areas with slower cooling rates and the absence of a well-defined skin.
- Higher cooling rates promote skin formation and reduce soldering.
The study showed that solder can be mitigated through careful control of alloy composition and process parameters.
Possible areas for future expansion research:
- Further investigation of the skin-matrix interface and its role in failure.
- Quantitative analysis of the skin's mechanical properties at elevated temperatures.
- Exploration of other alloying elements and their effects on skin formation and high-temperature strength.
- Development of more accurate predictive models for skin thickness and soldering behavior.
- Optimization of die design and cooling strategies to promote uniform skin formation.
8. References:
- [1] S. P. Udvardy, "2020 State of the Die Casting Industry," Die Casting Engineer, pp. 10-13, Janurary 2021.
- [2] J. A. Lee, "Cast Aluminum Alloy for High Temperature Applications," in The 132nd TMS Annual Meeting & Exhibition, San Diego, 2003.
- [3] D. Schwam and A. Miller, "Thin-Wall and High-Strength Die Casting Alloys," Defense Technical Information Center, 2017.
- [4] S. Aoyama, M. Akase, K. Sakamoto and T. Umemura, "Die Lubricant Deposit and Its Effect on Ejection in Die Casting," in NADCA Congress and Exposition, Detroit, 1991.
- [5] D. J. Blondheim, Jr., "System Understanding of High Pressure Die Casting Process and Data with Machine Learing Applications," Colorado State University, Fort Collins, CO, 2021.
- [6] R. L. Lewis and C. L. Chen, "Progress Report: Development of an Accelerated Testing Procedure for Die Casting Die Wear Analysis," NSF Engineering Research Center for Net Shape Manufacturing, 1989.
- [7] C. Tang, M. Z. Jahedi and M. Brandt, "Investigation of the Soldering Reaction in Magnesium High Pressure Die Casting Dies," in 6TH INTERNATIONAL TOOLING CONFERENCE, Karlstad, Sweden, 2002.
- [8] D. Argo, R. J. Barnhurst and W. Walkington, "NADCA Sponsored Research: The Causes of Soldering in Zinc Die Casting," in NADCA Die Casting Congress, Minneapolis, 1997.
- [9] A. Monroe and P. Sanders, "The Need for a New Approach to Soldering in High Pressure Die Casting," International Journal of Metalcasting, vol. 15, pp. 391-397, 2021.
- [10] B. Wang, "An Investigation of the Adhesion Behavior of Aluminum on Various PVD Coatings Applied to H13 Tool Steel to Minimize or Eliminate Lubrication During High Pressure Die Casting," Colorado School of Mines, 2016.
- [11] A. K. Monroe, Thermomechanical Mechanisms That Cause Adhesion of Aluminum High Pressure Die Castings to the Die, Houghton: Michigan Technological University, 2021.
- [12] M. Stern, "Progress of Die-Casting Industry," in Transactions of the American Foundrymen's Association, Cleveland, OH, 1930.
- [13] D. L. Colwell and E. Trela, "New Aluminum Die Casting Alloys," in Transactions of the American Foundrymen's Society, Atlantic City, NJ, 1954.
- [14] E. K. Holz, "Trouble Shooting Aluminum Die Casting Quality Problems," in 7th SDCE International Die Casting Congress, Chicago, IL, 1972.
- [15] W. Jonsson, "The Effect of Iron and Magnesium on the Properites of Aluminum Die Casting Alloys," p. 8, November 1964.
- [16] H. Tosa and A. Urakami, "Factors Influencing Die Casting Ejection," in North American Die Casting Congress, Chicago, IL, 1972.
- [17] W. Bonsack, "Trends in Aluminum Casting Alloys," Transactions of the American Foundrymen's Society, vol. 76, pp. 69-75, 1950.
- [18] D. L. Colwell and O. Tichy, "Machinability of Aluminum Die Castings," Transactions of the American Foundrymen's Society, vol. 92, pp. 236-241, 1956.
- [19] W. Bonsack, "Iron-The Problematic Factor in Quality Of Aluminum Alloy Die Castings," Transactions of the American Foundrymen's Society, vol. 114, pp. 712-720, 1961.
- [20] W. Kajoch and A. Fajkiel, "Testing the Soldering Tendencies of Aluminum Die Casting Alloy," in North American Die Casting Congress, Detroit, MI, 1991.
- [21] Y. L. Chu, P. Cheng and R. Shivpuri, "Soldering Phenomenon in Aluminum Die Casting: Possible Causes and Cures," in North American Die Casting Congress, Cleveland, 1993.
- [22] K. Bouché, F. Barbier and A. Coulet, "Intermetallic compound layer growth between solid iron and molten aluminium," Materials Science and Engineering A, vol. 249, pp. 167-175, 1998.
- [23] A. Bouayad, C. Gerometta, A. Belkebir and A. Ambari, "Kinetic interactions between solid iron and molten aluminium," Materials Science and Engineering A, vol. 363, pp. 53-61, 2003.
- [24] F. C. Yin, M.-x. Zhao, Y.-x. Liu, W. Han and Z. Li, "Effect of Si on growth kinetics of intermetallic compounds during reaction between solid iron and molten aluminum," Transactions of Nonferrous Metals Society of China, vol. 23, pp. 556-561, 2013.
- [25] H. Azimaee, M. Sarfaraz, M. Mirjalili and K. Aminian, "Effect of silicon and manganese on the kinetics and morphology of the intermetallic layer growth during hot-dip aluminizing," Surface & Coatings Technology, vol. 357, pp. 483-496, 2019.
- [26] K. Wladyslaw and F. Alexander, in Transactions of the North American Die Casting Association (NADCA) Congress and Expo, Rosemont, 1993.
- [27] S. Shankar and D. Apelian, "Die Soldering: Mechanism of the Interface Reaction between Molten Aluminum Alloy and Tool Steel," Metallurgical and Materials Transactions B, vol. 33, pp. 465-476, June 2002.
- [28] P. Terek, L. Kovačević, A. Miletić, P. Panjan, S. Baloš, B. Škorić and D. Kakaš, "Effects of die core treatments and surface finishes on the sticking and galling tendency of Al–Si alloy casting during ejection," Wear, Vols. 356-357, pp. 122-134, June 2016.
- [29] Z. W. Chen, "Formation and progression of die soldering during high pressure die casting," Materials Science and Engineering A, vol. 397, pp. 356-369, 2005.
- [30] S. Gulizia, M. Z. Jahedi and E. D. Doyle, "Performance evaluation of PVD coatings for high pressure die casting," Surface and Coatings Technology, vol. 140, no. 3, pp. 200-205, 1 June 2001.
- [31] Z. Chen and M. Z. Jahedi, "Die erosion and its effect on soldering formation in high pressure die casting of aluminium alloys," Materials & Design, vol. 20, no. 6, pp. 303-309, 9 September 1999.
- [32] Q. Han, E. A. Kenik and S. Viswanathan, "Die Soldering in Aluminum Die Casting," Oak Ridge, TN, 2000.
- [33] M. Kohlhepp, P. J. Uggowitzer, M. Hummel and H. W. Höppel, "Formation of Die Soldering and the Influence of Alloying Elements on the Intermetallic Interface," Materials, vol. 14, p. 1580, 24 March 2021.
- [34] C.-Y. Jeong, Y.-S. Kim, J.-H. Ryu and H.-J. Kim, "Mechanical and Die Soldering Properties of Al-Si-Mg Alloys with Vacuum HPDC Process," in 12th International Conference on Aluminium Alloys, Yokohama, Japan, 2010.
- [35] B. Wang, G. R. Bourne, A. L. Korenyi Both, A. K. Monroe, S. P. Midson and M. J. Kaufman, "Method to evaluate the adhesion behavior of aluminum-based alloyson various materials and coatings for lube-free die casting," Journal of Materials Processing Technology, vol. 237, pp. 386-393, 21 June 2016.
- [36] Y. Zhu, D. Schwam, J. F. Wallace and S. Birceanu, "Evaluation of soldering, washout and thermal fatigue resistance of advanced metal materials for aluminum die-casting dies," Materials Science and Engineering A, vol. 379, pp. 420-431, 2004.
- [37] Y. L. Chu, S. Balasubramaniam, R. Rajan and R. Shivpuri, "A Study of the Cast Alloy-Die Surface Interactions in Aluminum Die Casting," in North American Die Casting Congress, Minneapolis, MN, 1997.
- [38] K. Bobzin, T. Brögelmann, U. Hartmann and N. C. Kruppe, "Analysis of CrN/AlN/Al2O3 and two industrially used coatings deposited on die casting cores after application in an aluminum die casting machine," Surface and Coatings Technology, vol. 308, pp. 374-382, 25 December 2016.
- [39] Q. Han and S. Viswanathan, "Analysis of the mechanism of die soldering in aluminum die casting," Metallurgical and Materials Transactions A, vol. 34, pp. 139-146, January 2003.
- [40] S. Shankar and D. Apelian, "Mechanism and Preventive Measures for Die Soldering during Al Casting in a Ferrous Mold," JOM, vol. 54, pp. 47-54, August 2002.
- [41] L. A. Norstrom, B. Klarenfjord and M. Svensson, "General Aspects on ‘Washout’ Mechanisms in Aluminum Die Casting Dies," in North American Die Casting Congress, Cleveland, OH, 1993.
- [42] M. Yu, R. Shivpuri and R. A. Rapp, "Effects of molten aluminum on H13 dies and coatings," Journal of Materials Engineering and Performance, vol. 4, pp. 175-181, 1 April 1995.
- [43] Z. Chen, D. T. Fraser and M. Z. Jahedi, "Structures of intermetallic phases formed during immersion of H13 tool steel in an Al–11Si–3Cu die casting alloy melt," Materials Science and Engineering: A, vol. 260, no. 1-2, pp. 188-196, February 1999.
- [44] J. Song, X. Wang, T. DenOuden and Q. Han, "Evolution of Intermetallic Phases in Soldering of the Die Casting of Aluminum Alloys," Metallurgical and Materials Transactions A, vol. 47, pp. 2609-2615, 22 March 2016.
- [45] A. Duarte, F. J. Olivira and F. M. Costa, "Characterisation of interface formed at 650°C between AISI H13 steel and Al–12Si–1Cu aluminium melt," International Journal of Cast Metals Research, vol. 23, no. 4, pp. 231-239, 2010.
- [46] S. Sumanth and A. Diran, "The Role of Aluminum Alloy Chemistry and Die Material on Die Soldering," in North American Die Casting Congress, Cleveland, OH, 1999.
- [47] N. A. Belov, D. G. Eskin and A. A. A, Iron in Aluminium Alloys: Impurity and Alloying Element, Boca Raton: Taylor & Francis Group, 2002.
- [48] A. Cuevas, K. J. Schumann, C. J. Simpson and V. A. Ravi, "Aluminizing Of Stainless Steel," in CORROSION 2012, Salt Lake City, 2012.
- [49] W.-J. Cheng and C.-J. Wang, "Growth of intermetallic layer in the aluminide mild steel during hot-dipping," Surface & Coatings Technology, vol. 204, pp. 824-828, 2009.
- [50] T. Heumann and N. Dittrich, "Structure character of the Fe2Al5 intermetallic compound in hot dip aluminizing process," Z Metallk, vol. 50, pp. 617-623, 1959.
- [51] C. Tang, M. Jahedi and M. Brandt, "Role of Manganese in the Soldering Reaction in Magnesium High Pressure Die Casting," Advanced Engineering Materials, vol. 5, no. 12, pp. 829-927, December 2003.
- [52] C. Tang, "Soldering in Magnesium High Pressure Die Casting and its Prevention by Surface Engineering," Hawthorn, Victoria Australia , 2007.
- [53] A. E. Weatherill, "The Die Surface (Bloom and Calcite Layers) and Galvanizing (Cause and Prevention)," Zinc Development Association, London, 1985.
- [54] J. M. Birch, S. E. Booth and T. B. Hill, "Prevention of Soldering with Surface Coating of Dies," in NADCA International Die Casting Congress, 1991.
- [55] ASTM International, Standard Specification for Aluminum-Alloy Die Castings, 18 ed., West Conshohocken, PA, 2018.
- [56] D. R. Gunasegaram, B. R. Finnin and F. B. Polivka, "Melt flow velocity in high pressure die casting:its effect on microstructure and mechanical properties in an Al–Si alloy," Materials Science and Technology, vol. 23, no. 7, pp. 847-856, 19 July 2013.
- [57] M. M. Makhlouf, D. Apelian and L. Wang, "Microstructure and Properties of Aluminum Die Casting Alloys," North American Die Casting Association, Rosemont, 1998.
- [58] H. Y. Hunsicker, L. F. Mondolfo and P. A. Tomblin, "Properties of Pure Metals," in Properties and Selection: Nonferrous Alloys and Special-Purpose Materials, vol. 2, Materials Park, OH: ASM International, 1990, pp. 1099-1178.
- [59] L. Pauling, "Atomic Radii dnd Interatomic Distances in Metals," Journal of the American Chemical Society, vol. 69, no. 3, pp. 542-553, 1 March 1947.
- [60] K. A. Gschneidner, Jr. and M. Verkade, "Electronic and crystal structures, size (ECS2) model for predicting binary solid solutions," Progress in Materials Science, vol. 49, no. 3-4, pp. 411-428, 2004.
- [61] J. Yaokawa, Y. Iwata, N. Amano and T. Nakamichi, "Undercooled Solidification Analysis of Al-Si Eutectic Alloy with Consideration of Solidification Rate," Journal of Japan Foundry Engineering Society, vol. 84, no. 4, pp. 185-192, 25 April 2012.
- [62] J. G. Kaufman and E. L. Rooy, Aluminum Alloy Castings: Properties, Processes, and Applications, ASM International, 2004.
- [63] Z. Liu and V. Mohles, "Solution strengthening of various elements in aluminium alloys," Materials Science Forum, Vols. 794-796, pp. 473-478, 2014.
- [64] D. J. Lloyd, "The Work Hardening of Some Commercial Al Alloys," Materials Science Forum, vol. 519/521, pp. 55-62, 2006.
- [65] I. R. McAdams and Q. Han, "Effect of Cooling Conditions on Silafont 36 Phase Formation and Secondary Dendrite Arm Spacing," in NADCA Die Casting Congress, Atlanta, GA, 2017.
- [66] K. Ahmmed and H. Hu, "Effect of thermal treatment on tensile properties of vacuum die cast modified aluminum alloy A356," in NADCA Die Casting Congress, 2010.
- [67] X. Yan and F. Caron, "A New High Performance HPDC Aluminum Alloy," in NADCA Die Casting Congress, Indianapolis, IN, 2018.
- [68] G. Asghar, L. Peng, P. Fu, L. Yuan and Y. Liu, "Role of Mg2Si precipitates size in determining the ductility of A357 cast alloy," Materials & design, vol. 186, p. 108280, 2020.
- [69] A. K. Gupta, D. J. Lloyd and S. A. Court, "Precipitation hardening in Al–Mg–Si alloys with and without excess Si," Materials Science and Engineering A, vol. 316, pp. 11-17, November 2001.
- [70] A. Fortini, M. Merlin, E. Fabbri, S. Pirletti and G. L. Garagnani, "On the influence of Mn and Mg additions on tensile properties, microstructure and quality index of the A356 aluminum foundry alloy," Procedia Structural Integrity, vol. 2, pp. 2238-2245, 2016.
- [71] M. Yildirim and D. Özyürek, "The effects of Mg amount on the microstructure and mechanical properties of Al–Si–Mg alloys," Materials & Design, vol. 51, pp. 767-774, October 2013.
- [72] J. Hwang, R. Banerjee, H. W. Doty and M. J. Kaufman, "The effect of Mg on the structure and properties of Type 319 aluminum casting alloys," Acta Materialia, vol. 57, no. 4, pp. 1308-1317, February 2009.
- [73] R. J. Donahue, T. M. Cleary and K. R. Anderson, "HYPOEUTECTIC ALUMINUM-SILICON ALLOY HAVING REDUCED MICROPOROSITY". United States of America Patent 6923935, 2 August 2005.
- [74] R. Mackay and G. Byczynski, "Scaling Back Heat Treatment of Aluminum 319 Without Changing Material Properties," Modern Casting, pp. 28-33, 12 February 2024.
- [75] D. Bolibruchova and R. Podprocká, "The Effect of Different Mn/Fe Ratio on Microstructure Alloy Based on Al-Si-Mg," Archives of Foundry Engineering, vol. 19, no. 3, pp. 15-20, 2019.
- [76] W. Bonsack, "High Strength Nonheat-Treated Aluminum Casting Alloys," Transactions of the American Foundrymen's Society, vol. 100, pp. 453-459, 1952.
- [77] R. Lumley, R. G. Donnell, D. Gunasegaram, T. Kittel-Sherri, M. Gershenzon, A. C. Yob and I. J. Polmear, "The role of alloy composition in the heat treatment of aluminum high pressure die castings," Metallurgical Science and Technology, vol. 26, no. 2, pp. 1-11, Janurary 2008.
- [78] R. J. Donahue, "Proposed "Environmentally Green" High Pressure Die Casting Alloys with Low Iron for Improved Mechanical Properties and Strontium for Die Soldering Resistance," in NADCA Die Casting Congress, Columbus, OH, 2011.
- [79] K. Domkin, J. H. Hattel and J. Thorborg, "Modeling of high temperature- and diffusion-controlled die soldering in aluminum high pressure die casting," Journal of Materials Processing Technology, vol. 209, no. 8, pp. 4051-4061, 21 April 2009.
- [80] S.-B. Kim, Y.-H. Yim and D.-H. Roh, "Prediction of Die Soldering in Die Casting by a Numerical Algorithm based on the Combination of Thermal and Fluid Factors Verified with Experimental Results," in Die Casting Congress and Tabletop, Cleveland, OH, 2019.
- [81] R. Bhola and G. Muller, "Eliminating Die Solder with Pinpoint Accuracy Using Simulations," in Die Casting Congress & Tabletop, Cleveland, OH, 2019.
- [82] F. Widerøe and T. Welo, "Conditions for sticking friction between aluminium alloy AA6060 and tool steel in hot forming," Key Engineering Materials, vol. 491, pp. 121-128, 2012.
- [83] R. G. Budynas and J. K. Nisbett, Mechanical Engineering Design, 11 ed., McGraw-Hill, 2020.
- [84] M. Suzuki and K. Furumoto, "Influence of mass effect on physical and mechanical properties of ADC12 alloy die castings," Journal of Japan Institute of Light Metals, vol. 21, no. 1, pp. 36-45, 30 January 1971.
- [85] I. N. Khan, M. J. Starink and J. L. Yan, "A model for precipitation kinetics and strengthening in Al–Cu–Mg alloys," Materials Science and Engineering: A, vol. 472, no. 1-2, pp. 66-74, January 2008.
- [86] A. Kelly and K. M. Knowles, Crysallography and Crystal Defects, Second ed., John Wiley & Sons, Ltd, 2012.
- [87] E. Schmid and G. Siebel, Z. Elektrochem, vol. 37, p. 447, 1931.
- [88] M. E. Kassner, M. Z. Wang, M. T. Perez-Prado and S. Alhajeri, "Large-Strain Softening of Aluminum in Shear at Elevated Temperature," Metallurgical and Materials Transactions A, vol. 33A, pp. 3145-3153, October 2002.
- [89] H. Yang, S. Ji, W. Yang, Y. Wang and Z. Fan, "Effect of Mg level on the microstructure and mechanical properties of die-cast Al–Si–Cu alloys," Materials science & engineering A, vol. 642, pp. 340-350, 2015.
- [90] A. L. Kearney, "Properties of Cast Aluminum Alloys," in Properties and Selection: Nonferrous Alloys and Special-Purpose Materials, Vol 2, ASM International, 1990, p. 152–177.
- [91] W. J. M. Tegart, Elements of Mechanical Metallurgy, New York: Macmillan, 1966.
- [92] R. W. Hertzberg, R. P. Vinci and J. L. Hertzberg, Deformation and Fracture Mechanics of Engineering Materials, John Wiley & Sons Ltd, 2012.
- [93] C. Gu, Y. Lu, E. Cinkilic, J. Miao, A. Klarner, X. Yan and A. A. Luo, "Predicting grain structure in high pressure die casting of aluminum alloys: A coupled cellular automaton and process model," Computational Materials Science, vol. 161, pp. 64-75, 15 April 2019.
- [94] P. N. Nakashima, "The Crystallography of Aluminum and Its Alloys," in Encyclopedia of Aluminum and Its Alloys, G. E. Totten, Ed., Boca Raton, CRC Press, 2018, pp. 488-586.
- [95] H. B. Gray, Chemical Bonds: An Introduction to Atomic and Molecular Structure, Mill Valley, CA: University Science Books, 1994.
- [96] O. R. Myhr, Ö. Grong and S. J. Andersen, "Modelling of the age hardening behaviour of Al–Mg–Si alloys," Acta Materialia, vol. 49, no. 1, pp. 65-75, 8 January 2001.
- [97] H. J. Frost and M. F. Ashby, "The f.c.c. Metals: Ni, Cu, Ag, Al, Pb and γ-Fe," in Deformation-Mechanism Maps, The Plasticity and Creep of Metals and Ceramics, Franklin Book Company, 1982, p. 166.
- [98] W. Li, J. Ma, H. Kou, J. Shao, X. Zhang, Y. Deng, Y. Tao and D. Fang, "Modeling the effect of temperature on the yield strength of precipitation strengthening Ni-base superalloys," International Journal of Plasticity, vol. 116, pp. 143-158, May 2019.
- [99] D. E. Laughlin, W. F. Miao, L. M. Karabin and D. J. Chakrabarti, "Effects of Cu and Mn content and processing on precipitation hardening behavior in Al-Mg-Si Cu alloy 6022," in TMS Annual Meeting, Warrendale, PA, 1998.
- [100] D. J. Chakrabarti and D. E. Laughlin, "Phase relations and precipitation in Al–Mg–Si alloys with Cu additions," Progress in Materials Science, vol. 49, no. 3-4, pp. 389-410, 2004.
- [101] M. E. Kassner, C. S. Campbell and R. Ermagan, "Large-Strain Softening of Aluminumin Shear at Elevated Temperature:Influence of Dislocation Climb," Metallurgical and Materials Transactions A, vol. 48A, p. 3971–3974, September 2017.
- [102] S. J. Anderson, H. W. Zandbergen, J. C. Jansen, C. Traeholt, U. H. Tundal and O. Reiso, "The crystal structure of the β″ phase in Al–Mg–Si alloys," Acta Materialia, vol. 46, no. 9, pp. 3283-3298, 22 May 1998.
- [103] M. H. Jacobs, "The structure of the metastable precipitates formed during ageing of an Al-Mg-Si alloy," The Philosophical Magazine: A Journal of Theoretical Experimental and Applied Physics, vol. 26, no. 1, pp. 1-13, 1972.
- [104] K. Matsuda, S. Ikeno, Y. Uetani and T. Sato, "Metastable phases in an Al-Mg-Si alloy containing copper," Metallurgical and Materials Transactions A, vol. 32, pp. 1293-1299, June 2001.
- [105] L. Arnberg and B. Aurivillius, "The Crystal Structure of Al(x)Cu2Mg(12-x)Si7, (h-AlCuMgSi)," Acta Chemica Scandinavica, vol. 34, pp. 1-5, 1980.
- [106] S. D. Dumolt, D. E. Laughlin and J. C. Williams, "Formation of a Modified b' Phase in Aluminum Alloy 6061," Scripta Metallurgica, vol. 18, pp. 1347-1350, 1984.
- [107] K. Teichmann, C. D. Marioara, S. J. Andersen and K. Marthinsen, "TEM study of β′ precipitate interaction mechanisms with dislocations and β′ interfaces with the aluminium matrix in Al–Mg–Si alloys," Materials Characterization, vol. 75, pp. 1-7, January 2013.
- [108] R. Vissers, M. A. Van Huis, J. C. Jansen, H. W. Zandbergen, C. D. Marioara and S. J. Andersen, "The crystal structure of the β′ phase in Al–Mg–Si alloys," Acta Materialia, vol. 55, no. 11, pp. 3815-3823, June 2007.
- [109] H. S. Hasting, A. G. Frøseth, S. J. Andersen, R. Vissers, J. C. Walmsley, C. D. Marioara, F. Danoix, W. Lefebvre and R. Holmestad, "Composition of β″ precipitates in Al–Mg–Si alloys by atom probe tomography and first principles calculations," Journal of Applied Physics, vol. 106, no. 12, pp. 123527-9, 15 December 2009.
- [110] C. Meengam, S. Chainarong and S. Sitthipong, "Investigation of shear strength and metallurgy on semi-solid metal 356 aluminium alloy with lap joint by friction stir spot welding," Engineering and Applied Science Research, vol. 49, no. 2, pp. 155-167, March 2022.
- [111] L. A. Giannuzzi and F. A. Stevie, "A review of focused ion beam milling techniques for TEM specimen preparation," Micron, vol. 30, no. 3, pp. 197-204, June 1999.
- [112] L. Giannuzzi and N. Smith, "TEM Specimen Preparation with Plasma FIB Xe+ Ions," Microscopy and Microanalysis, vol. 17, no. 52, pp. 646-647, 1 July 2011.
- [113] L. A. Giannuzzi, J. L. Drown, S. R. Brown, R. B. Irwin and F. A. Stevie, "Applications of the FIB lift-out technique for TEM specimen preparation," Microscopy Research and Technique, vol. 41, no. 4, pp. 285-290, 15 May 1998.
- [114] X. Zhong, A. C. Wade, P. J. Withers, X. Zhou, C. Cai, S. J. Haigh and G. M. Burke, "Comparing Xe+pFIB and Ga+FIB for TEM sample preparation of Al alloys: Minimising FIB-induced artefacts," Journal of Microscopy, vol. 282, no. 2, pp. 101-112, May 2021.
- [115] G. S. Wang, K. Liu and S. L. Wang, "Evolution of Elevated-Temperature Strength and Creep Resistance during Multi-Step Heat Treatments in Al-Mn-Mg Alloy," Materials, vol. 11, no. 7, pp. 1158-, July 2018.
- [116] İ. Şimşek and D. Özyürek, "Investigation of the effects of Mg amount on microstructure and wear behavior of Al-Si-Mg alloys," Engineering Science and Technology, an International Journal, vol. 22, no. 1, pp. 370-375, February 2019.
- [117] Y. Xiang, D. J. Srolovitz, L. -T. Cheng and W. E, "Level set simulations of dislocation-particle bypass mechanisms," Acta Materialia, vol. 52, pp. 1745-1760, 2004.
- [118] A. Garza-Delgado, K. Kabiri-Bamoradian and R. A. Miller, "Determination of Elevated-Temperature Mechanical Properties of an Aluminum A380.0 Die Casting Alloy in the As-Cast Condition," in NADCA CastExpo, Atlanta, GA, 2008.
- [119] ASTM International, Standard Specification for Aluminum-Alloy Permanent Mold Castings, 19 ed., West Conshohocken, PA, 2019.
- [120] N. L. Parthasarathi, U. Borah and S. K. Albert, "Correlation between coefficient of friction and surface roughness in dry sliding wear of AISI 316 L (N) stainless steel at elevated temperatures," Computer Modelling and New Technologies, vol. 17, no. 1, pp. 51-63, January 2013.
- [121] D. Bell, E. Bell, J. V. Gonzalez, E. Kessenich and S. Midson, "Recommended Surface Finishes for PVD Coated Die Casting Dies," DIE CASTING ENGINEER, pp. 14-16, September 2022.
- [122] M. A. Steicher, "The Dissolution of Aluminum in Sodium Hydroxide Solutions. II," Journal of The Electrochemical Society, vol.